Tumour-targeting bacteria engineered to fight cancer
Recent advances in targeted therapy and immunotherapy have once again raised the hope that a cure might be within reach for many cancer types. Yet, most late-stage cancers are either insensitive to the therapies to begin with or develop resistance later. Therapy with live tumour-targeting bacteria provides a unique option to meet these challenges. Compared with most other therapeutics, the effectiveness of tumour-targeting bacteria is not directly affected by the ‘genetic makeup’ of a tumour. Bacteria initiate their direct antitumour effects from deep within the tumour, followed by innate and adaptive antitumour immune responses. As microscopic ‘robotic factories’, bacterial vectors can be reprogrammed following simple genetic rules or sophisticated synthetic bioengineering principles to produce and deliver anticancer agents on the basis of clinical needs. Therapeutic approaches using live tumour-targeting bacteria can either be applied as a monotherapy or complement other anticancer therapies to achieve better clinical outcomes. In this Review, we summarize the potential benefits and challenges of this approach. We discuss how live bacteria selectively induce tumour regression and provide examples to illustrate different ways to engineer bacteria for improved safety and efficacy. Finally, we share our experience and insights on oncology clinical trials with tumour-targeting bacteria, including a discussion of the regulatory issues.
This is a preview of subscription content, access via your institution
Access options
Access Nature and 54 other Nature Portfolio journals
Get Nature+, our best-value online-access subscription
cancel any time
Subscribe to this journal
Receive 12 print issues and online access
206,07 € per year
only 17,17 € per issue
Buy this article
- Purchase on SpringerLink
- Instant access to full article PDF
Prices may be subject to local taxes which are calculated during checkout
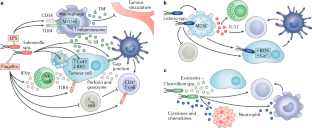
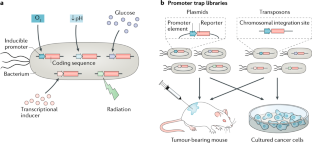
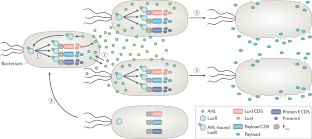
Similar content being viewed by others
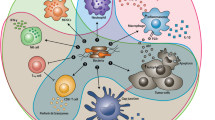
Bacteria-cancer interactions: bacteria-based cancer therapy
Article Open access 11 December 2019
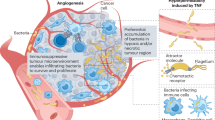
Exploiting bacteria for cancer immunotherapy
Article 05 June 2024
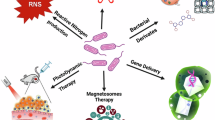
Bacterial nanotechnology as a paradigm in targeted cancer therapeutic delivery and immunotherapy
Article Open access 20 August 2024
References
- Chabner, B. A. & Roberts, T. G. Jr. Timeline: chemotherapy and the war on cancer. Nat. Rev. Cancer5, 65–72 (2005). CASPubMedGoogle Scholar
- Piccart-Gebhart, M. J. et al. Trastuzumab after adjuvant chemotherapy in HER2-positive breast cancer. N. Engl. J. Med.353, 1659–1672 (2005). CASPubMedGoogle Scholar
- Romond, E. H. et al. Trastuzumab plus adjuvant chemotherapy for operable HER2-positive breast cancer. N. Engl. J. Med.353, 1673–1684 (2005). CASPubMedGoogle Scholar
- Lynch, T. J. et al. Activating mutations in the epidermal growth factor receptor underlying responsiveness of non-small-cell lung cancer to gefitinib. N. Engl. J. Med.350, 2129–2139 (2004). CASPubMedGoogle Scholar
- Messersmith, W. A. & Ahnen, D. J. Targeting EGFR in colorectal cancer. N. Engl. J. Med.359, 1834–1836 (2008). CASPubMedGoogle Scholar
- Bollag, G. et al. Clinical efficacy of a RAF inhibitor needs broad target blockade in BRAF-mutant melanoma. Nature467, 596–599 (2010). CASPubMedPubMed CentralGoogle Scholar
- Flaherty, K. T. et al. Combined BRAF and MEK inhibition in melanoma with BRAF V600 mutations. N. Engl. J. Med.367, 1694–1703 (2012). CASPubMedPubMed CentralGoogle Scholar
- Widakowich, C. et al. Review: side effects of approved molecular targeted therapies in solid cancers. Oncologist12, 1443–1455 (2007). CASPubMedGoogle Scholar
- Lynch, T. J. Jr et al. Epidermal growth factor receptor inhibitor-associated cutaneous toxicities: an evolving paradigm in clinical management. Oncologist12, 610–621 (2007). CASPubMedGoogle Scholar
- Imai, K. & Takaoka, A. Comparing antibody and small-molecule therapies for cancer. Nat. Rev. Cancer6, 714–727 (2006). CASPubMedGoogle Scholar
- Rotow, J. & Bivona, T. G. Understanding and targeting resistance mechanisms in NSCLC. Nat. Rev. Cancer17, 637–658 (2017). CASPubMedGoogle Scholar
- Jones, S. et al. Personalized genomic analyses for cancer mutation discovery and interpretation. Sci. Transl Med.7, 283ra253 (2015).
- Coulie, P. G., Van den Eynde, B. J., van der Bruggen, P. & Boon, T. Tumour antigens recognized by T lymphocytes: at the core of cancer immunotherapy. Nat. Rev. Cancer14, 135–146 (2014). CASPubMedGoogle Scholar
- Topalian, S. L., Drake, C. G. & Pardoll, D. M. Immune checkpoint blockade: a common denominator approach to cancer therapy. Cancer Cell27, 450–461 (2015). CASPubMedPubMed CentralGoogle Scholar
- Postow, M. A., Callahan, M. K. & Wolchok, J. D. Immune checkpoint blockade in cancer therapy. J. Clin. Oncol.33, 1974–1982 (2015). CASPubMedPubMed CentralGoogle Scholar
- Rizvi, N. A. et al. Cancer immunology. Mutational landscape determines sensitivity to PD-1 blockade in non-small cell lung cancer. Science348, 124–128 (2015). CASPubMedPubMed CentralGoogle Scholar
- Le, D. T. et al. Mismatch-repair deficiency predicts response of solid tumors to PD-1 blockade. Science357, 409–413 (2017). CASPubMedPubMed CentralGoogle Scholar
- Tumeh, P. C. et al. PD-1 blockade induces responses by inhibiting adaptive immune resistance. Nature515, 568–571 (2014). CASPubMedPubMed CentralGoogle Scholar
- Yarchoan, M., Hopkins, A. & Jaffee, E. M. Tumor mutational burden and response rate to PD-1 inhibition. N. Engl. J. Med.377, 2500–2501 (2017). PubMedPubMed CentralGoogle Scholar
- Herbst, R. S. et al. Predictive correlates of response to the anti-PD-L1 antibody MPDL3280A in cancer patients. Nature515, 563–567 (2014). CASPubMedPubMed CentralGoogle Scholar
- Ribas, A. et al. Oncolytic virotherapy promotes intratumoral T cell infiltration and improves anti-PD-1 immunotherapy. Cell170, 1109–1119.e10 (2017). CASPubMedPubMed CentralGoogle Scholar
- Fang, J., Sawa, T. & Maeda, H. Factors and mechanism of “EPR” effect and the enhanced antitumor effects of macromolecular drugs including SMANCS. Adv. Exp. Med. Biol.519, 29–49 (2003). CASPubMedGoogle Scholar
- Jain, R. K. Normalizing tumor microenvironment to treat cancer: bench to bedside to biomarkers. J. Clin. Oncol.31, 2205–2218 (2013). This is an informative review on the abnormal tumour vasculature and microenvironment that are the anatomical foundation for bacterial therapy. CASPubMedPubMed CentralGoogle Scholar
- Fluegen, G. et al. Phenotypic heterogeneity of disseminated tumour cells is preset by primary tumour hypoxic microenvironments. Nat. Cell Biol.19, 120–132 (2017). CASPubMedPubMed CentralGoogle Scholar
- Brown, J. M. Tumor hypoxia in cancer therapy. Methods Enzymol.435, 297–321 (2007). CASPubMedGoogle Scholar
- Chouaib, S., Noman, M. Z., Kosmatopoulos, K. & Curran, M. A. Hypoxic stress: obstacles and opportunities for innovative immunotherapy of cancer. Oncogene36, 439–445 (2017). CASPubMedGoogle Scholar
- Mowday, A. M. et al. Advancing clostridia to clinical trial: past lessons and recent progress. Cancers (Basel)8, 63 (2016). Google Scholar
- Coley, W. B. I. I. Contribution to the knowledge of sarcoma. Ann. Surg.14, 199–220 (1891). CASPubMedPubMed CentralGoogle Scholar
- Coley, W. B. The treatment of malignant tumors by repeated inoculations of erysipelas. With a report of ten original cases. 1893. Clin. Orthop. Relat. Res. 3–11 (1991). This is a personal account by William B. Coley of some of the historical cases treated with live bacteria.
- Nauts, H. C., Swift, W. E. & Coley, B. L. The treatment of malignant tumors by bacterial toxins as developed by the late William B. Coley M. D., reviewed in the light of modern research. Cancer Res.6, 205–216 (1946). CASPubMedGoogle Scholar
- Forbes, N. S. Engineering the perfect (bacterial) cancer therapy. Nat. Rev. Cancer10, 785–794 (2010). This is a comprehensive Review on engineered tumour-targeting bacteria. CASPubMedPubMed CentralGoogle Scholar
- Bermudes, D., Zheng, L. M. & King, I. C. Live bacteria as anticancer agents and tumor-selective protein delivery vectors. Curr. Opin. Drug Discov. Dev.5, 194–199 (2002). CASGoogle Scholar
- Theys, J. et al. Tumor-specific gene delivery using genetically engineered bacteria. Curr. Gene Ther.3, 207–221 (2003). CASPubMedGoogle Scholar
- Chakrabarty, A. M. Microorganisms and cancer: quest for a therapy. J. Bacteriol.185, 2683–2686 (2003). CASPubMedPubMed CentralGoogle Scholar
- Minton, N. P. Clostridia in cancer therapy. Nat. Rev. Microbiol.1, 237–242 (2003). This is a comprehensive Review on using tumour-targetingClostridiumspp. for cancer therapy.CASPubMedGoogle Scholar
- Van Mellaert, L., Barbe, S. & Anne, J. Clostridium spores as anti-tumour agents. Trends Microbiol.14, 190–196 (2006). PubMedGoogle Scholar
- Hoffman, R. M. Tumor-seeking Salmonella amino acid auxotrophs. Curr. Opin. Biotechnol.22, 917–923 (2011). This is a comprehensive review on the development and efficacy of the amino acid auxotrophicS.Typhimurium A1-R strain, a majorSalmonellastrain being developed for cancer therapy.CASPubMedGoogle Scholar
- Lamm, D. L. BCG immunotherapy for transitional-cell carcinoma in situ of the bladder. Oncology (Williston Park)9, 947–952 discussion 955–965 (1995). CASGoogle Scholar
- Calmette, A. Preventive vaccination against tuberculosis with BCG. Proc. R. Soc. Med.24, 1481–1490 (1931). CASPubMedPubMed CentralGoogle Scholar
- Morales, A., Eidinger, D. & Bruce, A. W. Intracavitary bacillus Calmette-Guerin in the treatment of superficial bladder tumors. J. Urol.116, 180–183 (1976). CASPubMedGoogle Scholar
- De Jager, R. et al. Long-term complete remission in bladder carcinoma in situ with intravesical TICE bacillus Calmette Guerin. Overview analysis of six phase II clinical trials. Urology38, 507–513 (1991). PubMedGoogle Scholar
- Herr, H. W. et al. Intravesical bacillus Calmette-Guerin therapy prevents tumor progression and death from superficial bladder cancer: ten-year follow-up of a prospective randomized trial. J. Clin. Oncol.13, 1404–1408 (1995). CASPubMedGoogle Scholar
- Bohle, A., Gerdes, J., Ulmer, A. J., Hofstetter, A. G. & Flad, H. D. Effects of local bacillus Calmette-Guerin therapy in patients with bladder carcinoma on immunocompetent cells of the bladder wall. J. Urol.144, 53–58 (1990). CASPubMedGoogle Scholar
- Thalmann, G. N. et al. Urinary interleukin-8 and 18 predict the response of superficial bladder cancer to intravesical therapy with bacillus Calmette-Guerin. J. Urol.164, 2129–2133 (2000). CASPubMedGoogle Scholar
- Sharma, P., Old, L. J. & Allison, J. P. Immunotherapeutic strategies for high-risk bladder cancer. Semin. Oncol.34, 165–172 (2007). CASPubMedPubMed CentralGoogle Scholar
- Kasinskas, R. W. & Forbes, N. S. Salmonella typhimurium lacking ribose chemoreceptors localize in tumor quiescence and induce apoptosis. Cancer Res.67, 3201–3209 (2007). This study establishes that chemotaxis is involved in tumour targeting ofSalmonellaand that individual chemoreceptors guide the bacteria to different microenvironments.CASPubMedGoogle Scholar
- Pawelek, J. M., Low, K. B. & Bermudes, D. Tumor-targeted Salmonella as a novel anticancer vector. Cancer Res.57, 4537–4544 (1997). This study is the first to show thatSalmonellaspp. have tumour-targeting capacity.CASPubMedGoogle Scholar
- Diaz, L. A. Jr et al. Pharmacologic and toxicologic evaluation of C. novyi-NT spores. Toxicol. Sci.88, 562–575 (2005). This study showed that obligate anaerobeC. novyi-NT do not infect non-malignant hypoxic lesions.CASPubMedGoogle Scholar
- Min, J. J. et al. Noninvasive real-time imaging of tumors and metastases using tumor-targeting light-emitting Escherichia coli. Mol. Imaging Biol.10, 54–61 (2008). Google Scholar
- Weibel, S., Stritzker, J., Eck, M., Goebel, W. & Szalay, A. A. Colonization of experimental murine breast tumours by Escherichia coli K-12 significantly alters the tumour microenvironment. Cell. Microbiol.10, 1235–1248 (2008). CASPubMedGoogle Scholar
- Quispe-Tintaya, W. et al. Nontoxic radioactive Listeria(at) is a highly effective therapy against metastatic pancreatic cancer. Proc. Natl Acad. Sci. USA110, 8668–8673 (2013). CASPubMedPubMed CentralGoogle Scholar
- Lambin, P. et al. Colonisation of Clostridium in the body is restricted to hypoxic and necrotic areas of tumours. Anaerobe4, 183–188 (1998). This is one of the earliest studies to show thatClostridiumspp. can selectively colonize solid tumours.CASPubMedGoogle Scholar
- Clairmont, C. et al. Biodistribution and genetic stability of the novel antitumor agent VNP20009, a genetically modified strain of Salmonella typhimurium. J. Infect. Dis.181, 1996–2002 (2000). CASPubMedGoogle Scholar
- Yu, Y. A. et al. Visualization of tumors and metastases in live animals with bacteria and vaccinia virus encoding light-emitting proteins. Nat. Biotechnol.22, 313–320 (2004). This study shows tumour-selective accumulation of intravenously injected tumour-targeting bacteria in real time. CASPubMedGoogle Scholar
- Chandra, D., Jahangir, A., Quispe-Tintaya, W., Einstein, M. H. & Gravekamp, C. Myeloid-derived suppressor cells have a central role in attenuated Listeria monocytogenes-based immunotherapy against metastatic breast cancer in young and old mice. Br. J. Cancer108, 2281–2290 (2013). This study shows that MDSCs facilitate the targeting of aListeriasp. to both primary and metastatic tumours.CASPubMedPubMed CentralGoogle Scholar
- Chandra, D. et al. 32-phosphorus selectively delivered by listeria to pancreatic cancer demonstrates a strong therapeutic effect. Oncotarget8, 20729–20740 (2017). PubMedPubMed CentralGoogle Scholar
- Agrawal, N. et al. Bacteriolytic therapy can generate a potent immune response against experimental tumors. Proc. Natl Acad. Sci. USA101, 15172–15177 (2004). CASPubMedPubMed CentralGoogle Scholar
- Westphal, K., Leschner, S., Jablonska, J., Loessner, H. & Weiss, S. Containment of tumor-colonizing bacteria by host neutrophils. Cancer Res.68, 2952–2960 (2008). CASPubMedGoogle Scholar
- Lardner, A. The effects of extracellular pH on immune function. J. Leukoc. Biol.69, 522–530 (2001). CASPubMedGoogle Scholar
- Joyce, J. A. & Fearon, D. T. T cell exclusion, immune privilege, and the tumor microenvironment. Science348, 74–80 (2015). CASPubMedGoogle Scholar
- Staedtke, V. et al. Clostridium novyi-NT can cause regression of orthotopically implanted glioblastomas in rats. Oncotarget6, 5536–5546 (2015). PubMedPubMed CentralGoogle Scholar
- Yu, Y. A., Zhang, Q. & Szalay, A. A. Establishment and characterization of conditions required for tumor colonization by intravenously delivered bacteria. Biotechnol. Bioeng.100, 567–578 (2008). CASPubMedGoogle Scholar
- Freitag, N. E., Rong, L. & Portnoy, D. A. Regulation of the prfA transcriptional activator of Listeria monocytogenes: multiple promoter elements contribute to intracellular growth and cell-to-cell spread. Infect. Immun.61, 2537–2544 (1993). CASPubMedPubMed CentralGoogle Scholar
- Roberts, N. J. et al. Intratumoral injection of Clostridium novyi-NT spores induces antitumor responses. Sci. Transl Med.6, 249ra111 (2014). This study shows promising antitumour responses to intratumourally injectedC. novyi-NT spores in both canine and human clinical studies.PubMedPubMed CentralGoogle Scholar
- Chagnon, A., Hudon, C., McSween, G., Vinet, G. & Fredette, V. Cytotoxicity and reduction of animal cell growth by Clostridium M-55 spores and their extracts. Cancer29, 431–434 (1972). CASPubMedGoogle Scholar
- Middlebrook, J. L. & Dorland, R. B. Bacterial toxins: cellular mechanisms of action. Microbiol. Rev.48, 199–221 (1984). CASPubMedPubMed CentralGoogle Scholar
- Bettegowda, C. et al. The genome and transcriptomes of the anti-tumor agent Clostridium novyi-NT. Nat. Biotechnol.24, 1573–1580 (2006). CASPubMedGoogle Scholar
- Cheong, I. et al. A bacterial protein enhances the release and efficacy of liposomal cancer drugs. Science314, 1308–1311 (2006). CASPubMedGoogle Scholar
- Avogadri, F. et al. Cancer immunotherapy based on killing of Salmonella-infected tumor cells. Cancer Res.65, 3920–3927 (2005). This study suggests that cross-presentation of tumour antigens resulting from tumour infection bySalmonellaspp. is at least partially responsible for tumour eradication.CASPubMedGoogle Scholar
- Lee, C. H., Wu, C. L. & Shiau, A. L. Toll-like receptor 4 mediates an antitumor host response induced by Salmonella choleraesuis. Clin. Cancer Res.14, 1905–1912 (2008). CASPubMedGoogle Scholar
- Leschner, S. et al. Tumor invasion of Salmonella enterica serovar Typhimurium is accompanied by strong hemorrhage promoted by TNF-alpha. PLOS ONE4, e6692 (2009). PubMedPubMed CentralGoogle Scholar
- Lee, C. H., Hsieh, J. L., Wu, C. L., Hsu, P. Y. & Shiau, A. L. T cell augments the antitumor activity of tumor-targeting. Salmonella. Appl. Microbiol. Biotechnol.90, 1381–1388 (2011). CASPubMedGoogle Scholar
- Kaimala, S. et al. Salmonella-mediated tumor regression involves targeting of tumor myeloid suppressor cells causing a shift to M1-like phenotype and reduction in suppressive capacity. Cancer Immunol. Immunother.63, 587–599 (2014). CASPubMedGoogle Scholar
- Jahangir, A. et al. Immunotherapy with Listeria reduces metastatic breast cancer in young and old mice through different mechanisms. Oncoimmunology6, e1342025 (2017). PubMedPubMed CentralGoogle Scholar
- Zhang, X. et al. The genes slyA, STM3120 and htrA are required for the anticancer ability of VNP20009. Oncotarget7, 81187–81196 (2016). PubMedPubMed CentralGoogle Scholar
- Dang, L. H., Bettegowda, C., Huso, D. L., Kinzler, K. W. & Vogelstein, B. Combination bacteriolytic therapy for the treatment of experimental tumors. Proc. Natl Acad. Sci. USA98, 15155–15160 (2001). CASPubMedPubMed CentralGoogle Scholar
- Beutler, B. & Rietschel, E. T. Innate immune sensing and its roots: the story of endotoxin. Nat. Rev. Immunol.3, 169–176 (2003). CASPubMedGoogle Scholar
- Low, K. B. et al. Lipid A mutant Salmonella with suppressed virulence and TNFalpha induction retain tumor-targeting in vivo. Nat. Biotechnol.17, 37–41 (1999). This study shows that deletion of themsbBgene drastically reduced the toxicity of aSalmonellasp., which is the genetic basis for VNP20009, aSalmonellastrain widely used in the field of cancer therapy with tumour-targeting bacteria.CASPubMedGoogle Scholar
- Toso, J. F. et al. Phase I study of the intravenous administration of attenuated Salmonella typhimurium to patients with metastatic melanoma. J. Clin. Oncol.20, 142–152 (2002). This is one of the earliest US Food and Drug Administration-approved clinical trials with live tumour-targeting bacteria.PubMedGoogle Scholar
- Heimann, D. M. & Rosenberg, S. A. Continuous intravenous administration of live genetically modified salmonella typhimurium in patients with metastatic melanoma. J. Immunother.26, 179–180 (2003). PubMedPubMed CentralGoogle Scholar
- Low, K. B. et al. Construction of VNP20009: a novel, genetically stable antibiotic-sensitive strain of tumor-targeting Salmonella for parenteral administration in humans. Methods Mol. Med.90, 47–59 (2004). CASPubMedGoogle Scholar
- Na, H. S. et al. Immune response induced by Salmonella typhimurium defective in ppGpp synthesis. Vaccine24, 2027–2034 (2006). CASPubMedGoogle Scholar
- Jeong, J. H. et al. Salmonella enterica serovar gallinarum requires ppGpp for internalization and survival in animal cells. J. Bacteriol.190, 6340–6350 (2008). CASPubMedPubMed CentralGoogle Scholar
- Nguyen, V. H. et al. Genetically engineered Salmonella typhimurium as an imageable therapeutic probe for cancer. Cancer Res.70, 18–23 (2010). CASPubMedGoogle Scholar
- Paterson, Y., Guirnalda, P. D. & Wood, L. M. Listeria and Salmonella bacterial vectors of tumor-associated antigens for cancer immunotherapy. Semin. Immunol.22, 183–189 (2010). CASPubMedPubMed CentralGoogle Scholar
- Toussaint, B., Chauchet, X., Wang, Y., Polack, B. & Le Gouellec, A. Live-attenuated bacteria as a cancer vaccine vector. Expert Rev. Vaccines12, 1139–1154 (2013). CASPubMedGoogle Scholar
- Wood, L. M. & Paterson, Y. Attenuated Listeria monocytogenes: a powerful and versatile vector for the future of tumor immunotherapy. Front. Cell. Infect. Microbiol.4, 51 (2014). PubMedPubMed CentralGoogle Scholar
- Gunn, G. R. et al. Two Listeria monocytogenes vaccine vectors that express different molecular forms of human papilloma virus-16 (HPV-16) E7 induce qualitatively different T cell immunity that correlates with their ability to induce regression of established tumors immortalized by HPV-16. J. Immunol.167, 6471–6479 (2001). CASPubMedGoogle Scholar
- Singh, M. et al. Direct incorporation of the NKT-cell activator alpha-galactosylceramide into a recombinant Listeria monocytogenes improves breast cancer vaccine efficacy. Br. J. Cancer111, 1945–1954 (2014). CASPubMedPubMed CentralGoogle Scholar
- Brockstedt, D. G. et al. Listeria-based cancer vaccines that segregate immunogenicity from toxicity. Proc. Natl Acad. Sci. USA101, 13832–13837 (2004). CASPubMedPubMed CentralGoogle Scholar
- Deng, W. et al. Recombinant Listeria promotes tumor rejection by CD8(+) T cell-dependent remodeling of the tumor microenvironment. Proc. Natl Acad. Sci. USA115, 8179-8184 (2018). CASPubMedGoogle Scholar
- Zhao, M. et al. Tumor-targeting bacterial therapy with amino acid auxotrophs of GFP-expressing Salmonella typhimurium. Proc. Natl Acad. Sci. USA102, 755–760 (2005). CASPubMedPubMed CentralGoogle Scholar
- Thompson, R. J., Bouwer, H. G., Portnoy, D. A. & Frankel, F. R. Pathogenicity and immunogenicity of a Listeria monocytogenes strain that requires D-alanine for growth. Infect. Immun.66, 3552–3561 (1998). CASPubMedPubMed CentralGoogle Scholar
- Malmgren, R. A. & Flanigan, C. C. Localization of the vegetative form of Clostridium tetani in mouse tumors following intravenous spore administration. Cancer Res.15, 473–478 (1955). CASPubMedGoogle Scholar
- Moese, J. R. & Moese, G. Oncolysis by clostridia. I. Activity of Clostridium butyricum (M-55) and other nonpathogenic clostridia against the ehrlich carcinoma. Cancer Res.24, 212–216 (1964). CASPubMedGoogle Scholar
- Desgrosellier, J. S. & Cheresh, D. A. Integrins in cancer: biological implications and therapeutic opportunities. Nat. Rev. Cancer10, 9–22 (2010). CASPubMedPubMed CentralGoogle Scholar
- Park, S. H. et al. RGD peptide cell-surface display enhances the targeting and therapeutic efficacy of attenuated Salmonella-mediated cancer therapy. Theranostics6, 1672–1682 (2016). CASPubMedPubMed CentralGoogle Scholar
- Bereta, M. et al. Improving tumor targeting and therapeutic potential of Salmonella VNP20009 by displaying cell surface CEA-specific antibodies. Vaccine25, 4183–4192 (2007). CASPubMedPubMed CentralGoogle Scholar
- Massa, P. E., Paniccia, A., Monegal, A., de Marco, A. & Rescigno, M. Salmonella engineered to express CD20-targeting antibodies and a drug-converting enzyme can eradicate human lymphomas. Blood122, 705–714 (2013). CASPubMedGoogle Scholar
- Pinero-Lambea, C. et al. Programming controlled adhesion of E. coli to target surfaces, cells, and tumors with synthetic adhesins. ACS Synthet. Biol.4, 463–473 (2015). CASGoogle Scholar
- Galen, J. E. & Levine, M. M. Can a ‘flawless’ live vector vaccine strain be engineered? Trends Microbiol.9, 372–376 (2001). CASPubMedGoogle Scholar
- Minton, N. P. et al. Chemotherapeutic tumour targeting using clostridial spores. FEMS Microbiol. Rev.17, 357–364 (1995). This is one of the earliest studies to show thatClostridiumspp. can be used to deliver antitumour effectors.CASPubMedGoogle Scholar
- Fox, M. E. et al. Anaerobic bacteria as a delivery system for cancer gene therapy: in vitro activation of 5-fluorocytosine by genetically engineered clostridia. Gene Ther.3, 173–178 (1996). CASPubMedGoogle Scholar
- Saltzman, D. A. et al. Attenuated Salmonella typhimurium containing interleukin-2 decreases MC-38 hepatic metastases: a novel anti-tumor agent. Cancer Biother. Radiopharm.11, 145–153 (1996). CASPubMedGoogle Scholar
- Russmann, H. et al. Delivery of epitopes by the Salmonella type III secretion system for vaccine development. Science281, 565–568 (1998). CASPubMedGoogle Scholar
- Walker, B. J., Stan, G. V. & Polizzi, K. M. Intracellular delivery of biologic therapeutics by bacterial secretion systems. Expert Rev. Mol. Med.19, e6 (2017).
- Camacho, E. M., Mesa-Pereira, B., Medina, C., Flores, A. & Santero, E. Engineering Salmonella as intracellular factory for effective killing of tumour cells. Sci. Rep.6, 30591 (2016). CASPubMedPubMed CentralGoogle Scholar
- Din, M. O. et al. Synchronized cycles of bacterial lysis for in vivo delivery. Nature536, 81–85 (2016). This study shows that bacteria can be engineered with sophisticated gene circuits to achieve desired therapeutic effects and safety profiles.CASPubMedPubMed CentralGoogle Scholar
- Jeong, J. H. et al. Anti-tumoral effect of the mitochondrial target domain of Noxa delivered by an engineered Salmonella typhimurium. PLOS ONE9, e80050 (2014). PubMedPubMed CentralGoogle Scholar
- Hense, M. et al. Eukaryotic expression plasmid transfer from the intracellular bacterium Listeria monocytogenes to host cells. Cell. Microbiol.3, 599–609 (2001). CASPubMedGoogle Scholar
- Lee, C. H., Wu, C. L. & Shiau, A. L. Systemic administration of attenuated Salmonella choleraesuis carrying thrombospondin-1 gene leads to tumor-specific transgene expression, delayed tumor growth and prolonged survival in the murine melanoma model. Cancer Gene Ther.12, 175–184 (2005). CASPubMedGoogle Scholar
- Fu, W. et al. Synergistic antitumor efficacy of suicide/ePNP gene and 6-methylpurine 2′-deoxyriboside via Salmonella against murine tumors. Cancer Gene Ther.15, 474–484 (2008). CASPubMedGoogle Scholar
- Darji, A., zur Lage, S., Garbe, A. I., Chakraborty, T. & Weiss, S. Oral delivery of DNA vaccines using attenuated Salmonella typhimurium as carrier. FEMS Immunol. Med. Microbiol.27, 341–349 (2000). CASPubMedGoogle Scholar
- Ryan, R. M. et al. Bacterial delivery of a novel cytolysin to hypoxic areas of solid tumors. Gene Ther.16, 329–339 (2009). CASPubMedGoogle Scholar
- St Jean, A. T., Swofford, C. A., Panteli, J. T., Brentzel, Z. J. & Forbes, N. S. Bacterial delivery of Staphylococcus aureus alpha-hemolysin causes regression and necrosis in murine tumors. Mol. Ther.22, 1266–1274 (2014). Google Scholar
- Hong, H. et al. Targeted deletion of the ara operon of Salmonella typhimurium enhances L-arabinose accumulation and drives PBAD-promoted expression of anti-cancer toxins and imaging agents. Cell Cycle13, 3112–3120 (2014). CASPubMedPubMed CentralGoogle Scholar
- Jiang, S. N. et al. Engineering of bacteria for the visualization of targeted delivery of a cytolytic anticancer agent. Mol. Ther.21, 1985–1995 (2013). CASPubMedPubMed CentralGoogle Scholar
- Quintero, D., Carrafa, J., Vincent, L. & Bermudes, D. EGFR-targeted chimeras of pseudomonas ToxA released into the extracellular milieu by attenuated Salmonella selectively kill tumor cells. Biotechnol. Bioeng.113, 2698–2711 (2016). CASPubMedPubMed CentralGoogle Scholar
- Lim, D. et al. Anti-tumor activity of an immunotoxin (TGFalpha-PE38) delivered by attenuated Salmonella typhimurium. Oncotarget8, 37550–37560 (2017). PubMedPubMed CentralGoogle Scholar
- Hersh, E. M. et al. Phase II studies of recombinant human tumor necrosis factor alpha in patients with malignant disease: a summary of the Southwest Oncology Group experience. J. Immunother.10, 426–431 (1991). CASPubMedGoogle Scholar
- Rensing-Ehl, A. et al. Local Fas/APO-1 (CD95) ligand-mediated tumor cell killing in vivo. Eur. J. Immunol.25, 2253–2258 (1995). CASPubMedGoogle Scholar
- Kelley, S. K. et al. Preclinical studies to predict the disposition of Apo2L/tumor necrosis factor-related apoptosis-inducing ligand in humans: characterization of in vivo efficacy, pharmacokinetics, and safety. J. Pharmacol. Exp. Ther.299, 31–38 (2001). CASPubMedGoogle Scholar
- Theys, J. et al. Stable Escherichia coli-Clostridium acetobutylicum shuttle vector for secretion of murine tumor necrosis factor alpha. Appl. Environ. Microbiol.65, 4295–4300 (1999). CASPubMedPubMed CentralGoogle Scholar
- Yoon, W. S., Chae, Y. S., Hong, J. & Park, Y. K. Antitumor therapeutic effects of a genetically engineered Salmonella typhimurium harboring TNF-alpha in mice. Appl. Microbiol. Biotechnol.89, 1807–1819 (2011). CASPubMedGoogle Scholar
- Loeffler, M., Le’Negrate, G., Krajewska, M. & Reed, J. C. Inhibition of tumor growth using salmonella expressing Fas ligand. J. Natl Cancer Inst.100, 1113–1116 (2008). CASPubMedPubMed CentralGoogle Scholar
- Ganai, S., Arenas, R. B. & Forbes, N. S. Tumour-targeted delivery of TRAIL using Salmonella typhimurium enhances breast cancer survival in mice. Br. J. Cancer101, 1683–1691 (2009). CASPubMedPubMed CentralGoogle Scholar
- Chen, J. et al. Salmonella-mediated tumor-targeting TRAIL gene therapy significantly suppresses melanoma growth in mouse model. Cancer Sci.103, 325–333 (2012). CASPubMedGoogle Scholar
- Zoaby, N. et al. Autonomous bacterial nanoswimmers target cancer. J. Control. Release257, 68–75 (2017). CASPubMedGoogle Scholar
- Zheng, D. W. et al. Optically-controlled bacterial metabolite for cancer therapy. Nat. Commun.9, 1680 (2018). PubMedPubMed CentralGoogle Scholar
- Austin, E. A. & Huber, B. E. A first step in the development of gene therapy for colorectal carcinoma: cloning, sequencing, and expression of Escherichia coli cytosine deaminase. Mol. Pharmacol.43, 380–387 (1993). CASPubMedGoogle Scholar
- King, I. et al. Tumor-targeted Salmonella expressing cytosine deaminase as an anticancer agent. Hum. Gene Ther.13, 1225–1233 (2002). CASPubMedGoogle Scholar
- Theys, J. et al. Repeated cycles of Clostridium-directed enzyme prodrug therapy result in sustained antitumour effects in vivo. Br. J. Cancer95, 1212–1219 (2006). CASPubMedPubMed CentralGoogle Scholar
- Knox, R. J., Friedlos, F. & Boland, M. P. The bioactivation of CB 1954 and its use as a prodrug in antibody-directed enzyme prodrug therapy (ADEPT). Cancer Metastasis Rev.12, 195–212 (1993). CASPubMedGoogle Scholar
- Palmer, D. H., Milner, A. E., Kerr, D. J. & Young, L. S. Mechanism of cell death induced by the novel enzyme-prodrug combination, nitroreductase/CB1954, and identification of synergism with 5-fluorouracil. Br. J. Cancer89, 944–950 (2003). CASPubMedPubMed CentralGoogle Scholar
- Lehouritis, P., Stanton, M., McCarthy, F. O., Jeavons, M. & Tangney, M. Activation of multiple chemotherapeutic prodrugs by the natural enzymolome of tumour-localised probiotic bacteria. J. Control. Release222, 9–17 (2016). CASPubMedGoogle Scholar
- Chen, G. et al. Tumor-targeting Salmonella typhimurium, a natural tool for activation of prodrug 6MePdR and their combination therapy in murine melanoma model. Appl. Microbiol. Biotechnol.97, 4393–4401 (2013). CASPubMedGoogle Scholar
- Saltzman, D. A. et al. Antitumor mechanisms of attenuated Salmonella typhimurium containing the gene for human interleukin-2: a novel antitumor agent? J. Pediatr. Surg.32, 301–306 (1997). CASPubMedGoogle Scholar
- Loeffler, M., Le’Negrate, G., Krajewska, M. & Reed, J. C. Attenuated Salmonella engineered to produce human cytokine LIGHT inhibit tumor growth. Proc. Natl Acad. Sci. USA104, 12879–12883 (2007). CASPubMedPubMed CentralGoogle Scholar
- Zheng, J. H. et al. Two-step enhanced cancer immunotherapy with engineered Salmonella typhimurium secreting heterologous flagellin. Sci. Transl Med.9, eaak9537 (2017). PubMedGoogle Scholar
- Binder, D. C. et al. Antigen-specific bacterial vaccine combined with anti-PD-L1 rescues dysfunctional endogenous T cells to reject long-established cancer. Cancer Immunol. Res.1, 123–133 (2013). CASPubMedPubMed CentralGoogle Scholar
- US National Library of Medicine. ClinicalTrials.gov https://clinicaltrials.gov/ct2/show/NCT03435952 (2018).
- Groot, A. J. et al. Functional antibodies produced by oncolytic clostridia. Biochem. Biophys. Res. Commun.364, 985–989 (2007). CASPubMedGoogle Scholar
- Naidoo, J. et al. Toxicities of the anti-PD-1 and anti-PD-L1 immune checkpoint antibodies. Ann. Oncol.26, 2375–2391 (2015). CASPubMedPubMed CentralGoogle Scholar
- Drake, C. G., Jaffee, E. & Pardoll, D. M. Mechanisms of immune evasion by tumors. Adv. Immunol.90, 51–81 (2006). CASPubMedGoogle Scholar
- Theys, J. et al. Improvement of Clostridium tumour targeting vectors evaluated in rat rhabdomyosarcomas. FEMS Immunol. Med. Microbiol.30, 37–41 (2001). CASPubMedGoogle Scholar
- Dang, L. H. et al. Targeting vascular and avascular compartments of tumors with C. novyi-NT and anti-microtubule agents. Cancer Biol. Ther.3, 326–337 (2004). CASPubMedGoogle Scholar
- Drees, J. J., Mertensotto, M. J., Augustin, L. B., Schottel, J. L. & Saltzman, D. A. Vasculature disruption enhances bacterial targeting of autochthonous tumors. J. Cancer6, 843–848 (2015). CASPubMedPubMed CentralGoogle Scholar
- Niethammer, A. G. et al. A DNA vaccine against VEGF receptor 2 prevents effective angiogenesis and inhibits tumor growth. Nat. Med.8, 1369–1375 (2002). CASPubMedGoogle Scholar
- Luo, Y., Markowitz, D., Xiang, R., Zhou, H. & Reisfeld, R. A. FLK-1-based minigene vaccines induce T cell-mediated suppression of angiogenesis and tumor protective immunity in syngeneic BALB/c mice. Vaccine25, 1409–1415 (2007). CASPubMedGoogle Scholar
- Lee, S. H. et al. Endoglin (CD105) is a target for an oral DNA vaccine against breast cancer. Cancer Immunol. Immunother.55, 1565–1574 (2006). CASPubMedGoogle Scholar
- Jarosz, M. et al. Therapeutic antitumor potential of endoglin-based DNA vaccine combined with immunomodulatory agents. Gene Ther.20, 262–273 (2013). CASPubMedGoogle Scholar
- Wood, L. M. et al. Targeting tumor vasculature with novel Listeria-based vaccines directed against CD105. Cancer Immunol. Immunother.60, 931–942 (2011). CASPubMedPubMed CentralGoogle Scholar
- Ruan, Z. et al. DNA vaccine against tumor endothelial marker 8 inhibits tumor angiogenesis and growth. J. Immunother.32, 486–491 (2009). CASPubMedGoogle Scholar
- Kaplan, C. D. et al. A novel DNA vaccine encoding PDGFRbeta suppresses growth and dissemination of murine colon, lung and breast carcinoma. Vaccine24, 6994–7002 (2006). CASPubMedGoogle Scholar
- Seavey, M. M., Maciag, P. C., Al-Rawi, N., Sewell, D. & Paterson, Y. An anti-vascular endothelial growth factor receptor 2/fetal liver kinase-1 Listeria monocytogenes anti-angiogenesis cancer vaccine for the treatment of primary and metastatic Her-2/neu + breast tumors in a mouse model. J. Immunol.182, 5537–5546 (2009). CASPubMedGoogle Scholar
- Manuel, E. R. et al. Salmonella-based therapy targeting indoleamine 2,3-dioxygenase coupled with enzymatic depletion of tumor hyaluronan induces complete regression of aggressive pancreatic tumors. Cancer Immunol. Res.3, 1096–1107 (2015). CASPubMedPubMed CentralGoogle Scholar
- Shizuya, H. et al. Cloning and stable maintenance of 300-kilobase-pair fragments of human DNA in Escherichia coli using an F-factor-based vector. Proc. Natl Acad. Sci. USA89, 8794–8797 (1992). CASPubMedPubMed CentralGoogle Scholar
- Gibson, D. G. et al. Creation of a bacterial cell controlled by a chemically synthesized genome. Science329, 52–56 (2010). CASPubMedGoogle Scholar
- Hutchison, C. A. III et al. Design and synthesis of a minimal bacterial genome. Science351, aad6253 (2016). PubMedGoogle Scholar
- Gardner, T. S., Cantor, C. R. & Collins, J. J. Construction of a genetic toggle switch in Escherichia coli. Nature403, 339–342 (2000). CASPubMedGoogle Scholar
- Elowitz, M. B. & Leibler, S. A synthetic oscillatory network of transcriptional regulators. Nature403, 335–338 (2000). CASPubMedGoogle Scholar
- Khalil, A. S. & Collins, J. J. Synthetic biology: applications come of age. Nat. Rev. Genet.11, 367–379 (2010). CASPubMedPubMed CentralGoogle Scholar
- Danino, T., Mondragon-Palomino, O., Tsimring, L. & Hasty, J. A synchronized quorum of genetic clocks. Nature463, 326–330 (2010). CASPubMedPubMed CentralGoogle Scholar
- Talmadge, J. E., Singh, R. K., Fidler, I. J. & Raz, A. Murine models to evaluate novel and conventional therapeutic strategies for cancer. Am. J. Pathol.170, 793–804 (2007). CASPubMedPubMed CentralGoogle Scholar
- Mignon, C., Sodoyer, R. & Werle, B. Antibiotic-free selection in biotherapeutics: now and forever. Pathogens4, 157–181 (2015). CASGoogle Scholar
- Martinez-Morales, F., Borges, A. C., Martinez, A., Shanmugam, K. T. & Ingram, L. O. Chromosomal integration of heterologous DNA in Escherichia coli with precise removal of markers and replicons used during construction. J. Bacteriol.181, 7143–7148 (1999). CASPubMedPubMed CentralGoogle Scholar
- Nemunaitis, J. et al. Pilot trial of genetically modified, attenuated Salmonella expressing the E. coli cytosine deaminase gene in refractory cancer patients. Cancer Gene Ther.10, 737–744 (2003). This is the first published human clinical study with the VNP20009 strain engineered to express an effector. CASPubMedGoogle Scholar
- Heap, J. T. et al. Spores of Clostridium engineered for clinical efficacy and safety cause regression and cure of tumors in vivo. Oncotarget5, 1761–1769 (2014). PubMedPubMed CentralGoogle Scholar
- Centers for Disease Control and Prevention. Active Bacterial Core surveillance (ABCs). CDChttps://www.cdc.gov/abcs/overview/background.html (2018).
- The United States Pharmacopeial Convention. Microbiological examination of nonsterile products. The United States Pharmacopeial Conventionhttps://hmc.usp.org/sites/default/files/documents/HMC/GCs-Pdfs/c62.pdf (2018).
- Kashiwagi, N. et al. Vascular supply with angio-CT for superselective intra-arterial chemotherapy in advanced maxillary sinus cancer. Br. J. Radiol.83, 171–178 (2010). CASPubMedPubMed CentralGoogle Scholar
- Fleming, I. N. et al. Imaging tumour hypoxia with positron emission tomography. Br. J. Cancer112, 238–250 (2015). CASPubMedGoogle Scholar
- Egeland, T. A., Gaustad, J. V., Galappathi, K. & Rofstad, E. K. Magnetic resonance imaging of tumor necrosis. Acta Oncol.50, 427–434 (2011). CASPubMedGoogle Scholar
- Bettegowda, C. et al. Imaging bacterial infections with radiolabeled 1-(2’-deoxy-2’-fluoro-beta-D-arabinofuranosyl)-5-iodouracil. Proc. Natl Acad. Sci. USA102, 1145–1150 (2005). CASPubMedPubMed CentralGoogle Scholar
- Diaz, L. A. Jr et al. Imaging of musculoskeletal bacterial infections by [124I]FIAU-PET/CT. PLOS ONE2, e1007 (2007). PubMedPubMed CentralGoogle Scholar
- Liu, G. et al. Noninvasive imaging of infection after treatment with tumor-homing bacteria using Chemical Exchange Saturation Transfer (CEST) MRI. Magn. Reson. Med.70, 1690–1698 (2013). CASPubMedGoogle Scholar
- Heppner, F. & Möse, J. R. The liquefaction (oncolysis) of malignant gliomas by a non pathogenic Clostridium. Acta Neurochir. (Wien)42, 123–125 (1978). CASGoogle Scholar
- Carey, R. W., Holland, J. F., Whang, H. Y., Neter, E. & Bryant, B. Clostridial oncolysis in man. Eur. J. Cancer3, 37–46 (1967). Google Scholar
- Vail, D. M. & MacEwen, E. G. Spontaneously occurring tumors of companion animals as models for human cancer. Cancer Invest.18, 781–792 (2000). CASPubMedGoogle Scholar
- Paoloni, M. & Khanna, C. Translation of new cancer treatments from pet dogs to humans. Nat. Rev. Cancer8, 147–156 (2008). CASPubMedGoogle Scholar
- Thamm, D. H. et al. Systemic administration of an attenuated, tumor-targeting Salmonella typhimurium to dogs with spontaneous neoplasia: phase I evaluation. Clin. Cancer Res.11, 4827–4834 (2005). This clinical study shows promising antitumour responses to the intravenously administered VNP20009 strain in canines.CASPubMedGoogle Scholar
- Fritz, S. E. et al. A phase I clinical study to evaluate safety of orally administered, genetically engineered Salmonella enterica serovar Typhimurium for canine osteosarcoma. Vet. Med. Sci.2, 179–190 (2016). This is the first published canine clinical study with an attenuatedSalmonellastrain engineered to express IL-2.CASPubMedPubMed CentralGoogle Scholar
- Le, D. T. et al. Safety and survival with GVAX pancreas prime and Listeria monocytogenes-expressing mesothelin (CRS-207) boost vaccines for metastatic pancreatic cancer. J. Clin. Oncol.33, 1325–1333 (2015). CASPubMedPubMed CentralGoogle Scholar
- Miles, B., Safran, H. P. & Monk, B. J. Therapeutic options for treatment of human papillomavirus-associated cancers - novel immunologic vaccines: ADXS11-001. Gynecol. Oncol. Res. Pract.4, 10 (2017). PubMedPubMed CentralGoogle Scholar
- US National Library of Medicine. ClinicalTrials.govhttp://www.clinicaltrials.gov/ct2/show/NCT00004216 (2013).
- US National Library of Medicine. ClinicalTrials.govhttp://www.clinicaltrials.gov/ct2/show/NCT00006254 (2013).
- US National Library of Medicine. ClinicalTrials.govhttp://www.clinicaltrials.gov/ct2/show/NCT00358397 (2016).
- US National Library of Medicine. ClinicalTrials.govhttp://www.clinicaltrials.gov/ct2/show/NCT00004988 (2008).
- US National Library of Medicine. ClinicalTrials.govhttp://www.clinicaltrials.gov/ct2/show/NCT01099631 (2017).
- US National Library of Medicine. ClinicalTrials.govhttp://www.clinicaltrials.gov/ct2/show/NCT01118819 (2016).
- US National Library of Medicine. ClinicalTrials.govhttp://www.clinicaltrials.gov/ct2/show/NCT01562626 (2017).
- US National Library of Medicine. ClinicalTrials.govhttp://www.clinicaltrials.gov/ct2/show/NCT01924689 (2018).
- Schmidt, W., Fabricius, E. M. & Schneeweiss, U. The tumour-Clostridium phenomenon: 50 years of developmental research (review). Int. J. Oncol.29, 1479–1492 (2006). CASPubMedGoogle Scholar
- Platt, J. et al. Antitumour effects of genetically engineered Salmonella in combination with radiation. Eur. J. Cancer36, 2397–2402 (2000). CASPubMedGoogle Scholar
- Bettegowda, C. et al. Overcoming the hypoxic barrier to radiation therapy with anaerobic bacteria. Proc. Natl Acad. Sci. USA100, 15083–15088 (2003). CASPubMedPubMed CentralGoogle Scholar
- Jiang, S. N. et al. Inhibition of tumor growth and metastasis by a combination of Escherichia coli-mediated cytolytic therapy and radiotherapy. Mol. Ther.18, 635–642 (2010). CASPubMedPubMed CentralGoogle Scholar
- Yano, S. et al. Tumor-targeting Salmonella typhimurium A1-R decoys quiescent cancer cells to cycle as visualized by FUCCI imaging and become sensitive to chemotherapy. Cell Cycle13, 3958–3963 (2014). CASPubMedPubMed CentralGoogle Scholar
- Igarashi, K. et al. Tumor-targeting Salmonella typhimurium A1-R combined with recombinant methioninase and cisplatinum eradicates an osteosarcoma cisplatinum-resistant lung metastasis in a patient-derived orthotopic xenograft (PDOX) mouse model: decoy, trap and kill chemotherapy moves toward the clinic. Cell Cycle17, 801–809 (2018). CASPubMedPubMed CentralGoogle Scholar
- U.S. Department of Health and Human Services. Recommendations for microbial vectors used for gene therapy. FDAhttps://www.fda.gov/downloads/Guidances/UCM466625.pdf (2016).
- U.S. Department of Health and Human Services. Preclinical assessment of investigational cellular and gene therapy products. FDAhttps://www.fda.gov/downloads/BiologicsBloodVaccines/GuidanceComplianceRegulatoryInformation/Guidances/CellularandGeneTherapy/UCM376521.pdf (2013).
- Uchugonova, A. et al. Imaging the different mechanisms of prostate cancer cell-killing by tumor-targeting Salmonella typhimurium A1-R. Anticancer Res.35, 5225–5229 (2015). CASPubMedGoogle Scholar
- Jia, L. J. et al. Oral delivery of tumor-targeting Salmonella for cancer therapy in murine tumor models. Cancer Sci.98, 1107–1112 (2007). CASPubMedGoogle Scholar
- Ganai, S., Arenas, R. B., Sauer, J. P., Bentley, B. & Forbes, N. S. In tumors Salmonella migrate away from vasculature toward the transition zone and induce apoptosis. Cancer Gene Ther.18, 457–466 (2011). CASPubMedPubMed CentralGoogle Scholar
- Lee, C. H. et al. Salmonella induce autophagy in melanoma by the downregulation of AKT/mTOR pathway. Gene Ther.21, 309–316 (2014). CASPubMedGoogle Scholar
- Zhou, S. et al. Suppression of pancreatic ductal adenocarcinoma growth by intratumoral delivery of attenuated Salmonella typhimurium using a dual fluorescent live tracking system. Cancer Biol. Ther.17, 732–740 (2016). CASPubMedPubMed CentralGoogle Scholar
- Kim, J. E. et al. Salmonella typhimurium suppresses tumor growth via the pro-inflammatory cytokine interleukin-1beta. Theranostics5, 1328–1342 (2015). CASPubMedPubMed CentralGoogle Scholar
- Phan, T. X. et al. Activation of inflammasome by attenuated Salmonella typhimurium in bacteria-mediated cancer therapy. Microbiol. Immunol.59, 664–675 (2015). CASPubMedGoogle Scholar
- Beutler, B. & Cerami, A. The biology of cachectin/TNF—a primary mediator of the host response. Annu. Rev. Immunol.7, 625–655 (1989). CASPubMedGoogle Scholar
- Dobrovolskaia, M. A. & Vogel, S. N. Toll receptors, CD14, and macrophage activation and deactivation by LPS. Microbes Infect.4, 903–914 (2002). CASPubMedGoogle Scholar
- Nguyen, C. T. et al. Flagellin enhances tumor-specific CD8( + ) T cell immune responses through TLR5 stimulation in a therapeutic cancer vaccine model. Vaccine31, 3879–3887 (2013). CASPubMedGoogle Scholar
- Sfondrini, L. et al. Antitumor activity of the TLR-5 ligand flagellin in mouse models of cancer. J. Immunol.176, 6624–6630 (2006). CASPubMedGoogle Scholar
- Cai, Z. et al. Activation of Toll-like receptor 5 on breast cancer cells by flagellin suppresses cell proliferation and tumor growth. Cancer Res.71, 2466–2475 (2011). CASPubMedPubMed CentralGoogle Scholar
- Leigh, N. D. et al. A flagellin-derived toll-like receptor 5 agonist stimulates cytotoxic lymphocyte-mediated tumor immunity. PLOS ONE9, e85587 (2014). PubMedPubMed CentralGoogle Scholar
- Kupz, A., Curtiss, R. III, Bedoui, S. & Strugnell, R. A. In vivo IFN-gamma secretion by NK cells in response to Salmonella typhimurium requires NLRC4 inflammasomes. PLOS ONE9, e97418 (2014). PubMedPubMed CentralGoogle Scholar
- Saccheri, F. et al. Bacteria-induced gap junctions in tumors favor antigen cross-presentation and antitumor immunity. Sci. Transl Med.2, 44ra57 (2010). PubMedGoogle Scholar
- Chang, W. W. et al. Salmonella enhance chemosensitivity in tumor through connexin 43 upregulation. Int. J. Cancer133, 1926–1935 (2013). CASPubMedGoogle Scholar
- Lin, H. C. et al. The inhibition of indoleamine 2, 3-dioxygenase 1 by connexin 43. Int. J. Med. Sci.14, 1181–1188 (2017). PubMedPubMed CentralGoogle Scholar
- Kim, S. H., Castro, F., Paterson, Y. & Gravekamp, C. High efficacy of a Listeria-based vaccine against metastatic breast cancer reveals a dual mode of action. Cancer Res.69, 5860–5866 (2009). This study shows thatListeriaspp. kill tumour cells through NADPH oxidase-mediated production of reactive oxygen species and intracellular calcium mobilization.CASPubMedPubMed CentralGoogle Scholar
- Mkrtichyan, M. et al. Anti-PD-1 antibody significantly increases therapeutic efficacy of Listeria monocytogenes (Lm)-LLO immunotherapy. J. Immunother. Cancer1, 15 (2013). PubMedPubMed CentralGoogle Scholar
- Wallecha, A., Singh, R. & Malinina, I. Listeria monocytogenes (Lm)-LLO immunotherapies reduce the immunosuppressive activity of myeloid-derived suppressor cells and regulatory T cells in the tumor microenvironment. J. Immunother.36, 468–476 (2013). CASPubMedGoogle Scholar
- Shinnoh, M. et al. Clostridium butyricum MIYAIRI 588 shows antitumor effects by enhancing the release of TRAIL from neutrophils through MMP-8. Int. J. Oncol.42, 903–911 (2013). CASPubMedGoogle Scholar
- Yu, B. et al. Explicit hypoxia targeting with tumor suppression by creating an “obligate” anaerobic Salmonella typhimurium strain. Sci. Rep.2, 436 (2012). PubMedPubMed CentralGoogle Scholar
- Flentie, K. et al. A bioluminescent transposon reporter-trap identifies tumor-specific microenvironment-induced promoters in Salmonella for conditional bacterial-based tumor therapy. Cancer Discov.2, 624–637 (2012). CASPubMedPubMed CentralGoogle Scholar
- Panteli, J. T. & Forbes, N. S. Engineered bacteria detect spatial profiles in glucose concentration within solid tumor cell masses. Biotechnol. Bioeng.113, 2474–2484 (2016). CASPubMedPubMed CentralGoogle Scholar
- Loessner, H. et al. Remote control of tumour-targeted Salmonella enterica serovar Typhimurium by the use of L-arabinose as inducer of bacterial gene expression in vivo. Cell. Microbiol.9, 1529–1537 (2007). CASPubMedGoogle Scholar
- Stritzker, J. et al. Tumor-specific colonization, tissue distribution, and gene induction by probiotic Escherichia coli Nissle 1917 in live mice. Int. J. Med. Microbiol.297, 151–162 (2007). CASPubMedGoogle Scholar
- Royo, J. L. et al. In vivo gene regulation in Salmonella spp. by a salicylate-dependent control circuit. Nat. Methods4, 937–942 (2007). CASPubMedGoogle Scholar
- Nuyts, S. et al. Increasing specificity of anti-tumor therapy: cytotoxic protein delivery by non-pathogenic clostridia under regulation of radio-induced promoters. Anticancer Res.21, 857–861 (2001). CASPubMedGoogle Scholar
- Nuyts, S. et al. Radio-responsive recA promoter significantly increases TNFalpha production in recombinant clostridia after 2 Gy irradiation. Gene Ther.8, 1197–1201 (2001). CASPubMedGoogle Scholar
- Nuyts, S. et al. The use of radiation-induced bacterial promoters in anaerobic conditions: a means to control gene expression in clostridium-mediated therapy for cancer. Radiat. Res.155, 716–723 (2001). CASPubMedGoogle Scholar
- Arrach, N., Zhao, M., Porwollik, S., Hoffman, R. M. & McClelland, M. Salmonella promoters preferentially activated inside tumors. Cancer Res.68, 4827–4832 (2008). This study identifiesSalmonellasp. promoter elements selectively active in the TME, which can be used for targeted colonization or payload expression.CASPubMedGoogle Scholar
- Leschner, S. et al. Identification of tumor-specific Salmonella typhimurium promoters and their regulatory logic. Nucleic Acids Res.40, 2984–2994 (2012). CASPubMedGoogle Scholar
- Swofford, C. A., St Jean, A. T., Panteli, J. T., Brentzel, Z. J. & Forbes, N. S. Identification of Staphylococcus aureus alpha-hemolysin as a protein drug that is secreted by anticancer bacteria and rapidly kills cancer cells. Biotechnol. Bioeng.111, 1233–1245 (2014). CASPubMedGoogle Scholar
- Zhang, H. Y. et al. Tumor-targeted delivery of biologically active TRAIL protein. Cancer Gene Ther.17, 334–343 (2010). CASPubMedPubMed CentralGoogle Scholar
- Zhang, Y. et al. Escherichia coli Nissle 1917 targets and restrains mouse B16 melanoma and 4T1 breast tumors through expression of azurin protein. Appl. Environ. Microbiol.78, 7603–7610 (2012). CASPubMedPubMed CentralGoogle Scholar
- Guan, G. F. et al. Salmonella typhimurium mediated delivery of Apoptin in human laryngeal cancer. Int. J. Med. Sci.10, 1639–1648 (2013). CASPubMedPubMed CentralGoogle Scholar
- Liu, S. C., Minton, N. P., Giaccia, A. J. & Brown, J. M. Anticancer efficacy of systemically delivered anaerobic bacteria as gene therapy vectors targeting tumor hypoxia/necrosis. Gene Ther.9, 291–296 (2002). CASPubMedGoogle Scholar
- Yi, C., Huang, Y., Guo, Z. Y. & Wang, S. R. Antitumor effect of cytosine deaminase/5-fluorocytosine suicide gene therapy system mediated by Bifidobacterium infantis on melanoma. Acta Pharmacol. Sin.26, 629–634 (2005). CASPubMedGoogle Scholar
- Fu, W., Lan, H., Liang, S., Gao, T. & Ren, D. Suicide gene/prodrug therapy using salmonella-mediated delivery of Escherichia coli purine nucleoside phosphorylase gene and 6-methoxypurine 2’-deoxyriboside in murine mammary carcinoma 4T1 model. Cancer Sci.99, 1172–1179 (2008). CASPubMedGoogle Scholar
- Stritzker, J., Pilgrim, S., Szalay, A. A. & Goebel, W. Prodrug converting enzyme gene delivery by L. monocytogenes. BMC Cancer8, 94 (2008). PubMedPubMed CentralGoogle Scholar
- Friedlos, F. et al. Attenuated Salmonella targets prodrug activating enzyme carboxypeptidase G2 to mouse melanoma and human breast and colon carcinomas for effective suicide gene therapy. Clin. Cancer Res.14, 4259–4266 (2008). CASPubMedGoogle Scholar
- Barak, Y. et al. New enzyme for reductive cancer chemotherapy, YieF, and its improvement by directed evolution. Mol. Cancer Ther.5, 97–103 (2006). CASPubMedGoogle Scholar
- Xiang, R. et al. An autologous oral DNA vaccine protects against murine melanoma. Proc. Natl Acad. Sci. USA97, 5492–5497 (2000). CASPubMedPubMed CentralGoogle Scholar
- Keenan, B. P. et al. A Listeria vaccine and depletion of T-regulatory cells activate immunity against early stage pancreatic intraepithelial neoplasms and prolong survival of mice. Gastroenterology146, 1784–1794.e6 (2014). CASPubMedGoogle Scholar
- Chou, C. K., Hung, J. Y., Liu, J. C., Chen, C. T. & Hung, M. C. An attenuated Salmonella oral DNA vaccine prevents the growth of hepatocellular carcinoma and colon cancer that express alpha-fetoprotein. Cancer Gene Ther.13, 746–752 (2006). CASPubMedGoogle Scholar
- Barbe, S. et al. Secretory production of biologically active rat interleukin-2 by Clostridium acetobutylicum DSM792 as a tool for anti-tumor treatment. FEMS Microbiol. Lett.246, 67–73 (2005). CASPubMedGoogle Scholar
- Agorio, C. et al. Live attenuated Salmonella as a vector for oral cytokine gene therapy in melanoma. J. Gene Med.9, 416–423 (2007). CASPubMedGoogle Scholar
- Yuhua, L. et al. Oral cytokine gene therapy against murine tumor using attenuated Salmonella typhimurium. Int. J. Cancer94, 438–443 (2001). CASPubMedGoogle Scholar
- Zhang, Y. L. et al. Clostridium sporogenes delivers interleukin-12 to hypoxic tumours, producing antitumour activity without significant toxicity. Lett. Appl. Microbiol.59, 580–586 (2014). CASPubMedGoogle Scholar
- Loeffler, M., Le’Negrate, G., Krajewska, M. & Reed, J. C. IL-18-producing Salmonella inhibit tumor growth. Cancer Gene Ther.15, 787–794 (2008). CASPubMedPubMed CentralGoogle Scholar
- Yoon, W. et al. Application of genetically engineered Salmonella typhimurium for interferon-gamma-induced therapy against melanoma. Eur. J. Cancer70, 48–61 (2017). CASPubMedGoogle Scholar
- Yoon, W. S., Choi, W. C., Sin, J. I. & Park, Y. K. Antitumor therapeutic effects of Salmonella typhimurium containing Flt3 Ligand expression plasmids in melanoma-bearing mouse. Biotechnol. Lett.29, 511–516 (2007). CASPubMedGoogle Scholar
- Loeffler, M., Le’Negrate, G., Krajewska, M. & Reed, J. C. Salmonella typhimurium engineered to produce CCL21 inhibit tumor growth. Cancer Immunol. Immunother.58, 769–775 (2009). CASPubMedGoogle Scholar
- Xiang, R., Luo, Y., Niethammer, A. G. & Reisfeld, R. A. Oral DNA vaccines target the tumor vasculature and microenvironment and suppress tumor growth and metastasis. Immunol. Rev.222, 117–128 (2008). CASPubMedGoogle Scholar
- Luo, Y. et al. Targeting tumor-associated macrophages as a novel strategy against breast cancer. J. Clin. Invest.116, 2132–2141 (2006). CASPubMedPubMed CentralGoogle Scholar
- Schmitz-Winnenthal, F. H. et al. A phase 1 trial extension to assess immunologic efficacy and safety of prime-boost vaccination with VXM01, an oral T cell vaccine against VEGFR2, in patients with advanced pancreatic cancer. Oncoimmunology7, e1303584 (2018). PubMedPubMed CentralGoogle Scholar
- Blache, C. A. et al. Systemic delivery of Salmonella typhimurium transformed with IDO shRNA enhances intratumoral vector colonization and suppresses tumor growth. Cancer Res.72, 6447–6456 (2012). CASPubMedPubMed CentralGoogle Scholar
- Zhang, L. et al. Intratumoral delivery and suppression of prostate tumor growth by attenuated Salmonella enterica serovar typhimurium carrying plasmid-based small interfering RNAs. Cancer Res.67, 5859–5864 (2007). CASPubMedGoogle Scholar
- Manuel, E. R. et al. Enhancement of cancer vaccine therapy by systemic delivery of a tumor-targeting Salmonella-based STAT3 shRNA suppresses the growth of established melanoma tumors. Cancer Res.71, 4183–4191 (2011). CASPubMedPubMed CentralGoogle Scholar
- Yang, N., Zhu, X., Chen, L., Li, S. & Ren, D. Oral administration of attenuated S. typhimurium carrying shRNA-expressing vectors as a cancer therapeutic. Cancer Biol. Ther.7, 145–151 (2008). CASPubMedGoogle Scholar
- Jiang, T. et al. Enhanced therapeutic effect of cisplatin on the prostate cancer in tumor-bearing mice by transfecting the attenuated Salmonella carrying a plasmid co-expressing p53 gene and mdm2 siRNA. Cancer Lett.337, 133–142 (2013). CASPubMedGoogle Scholar
- Liu, Y. B. et al. Plasmid-based Survivin shRNA and GRIM-19 carried by attenuated Salmonella suppresses tumor cell growth. Asian J. Androl.14, 536–545 (2012). CASPubMedPubMed CentralGoogle Scholar
- Li, Z. et al. Recombinant attenuated Salmonella typhimurium carrying a plasmid co-expressing ENDO-VEGI151 and survivin siRNA inhibits the growth of breast cancer in vivo. Mol. Med. Rep.7, 1215–1222 (2013). CASPubMedGoogle Scholar
- Jiang, Z. et al. Using attenuated Salmonella typhi as tumor targeting vector for MDR1 siRNA delivery. Cancer Biol. Ther.6, 555–560 (2007). CASPubMedGoogle Scholar
- Swofford, C. A., Van Dessel, N. & Forbes, N. S. Quorum-sensing Salmonella selectively trigger protein expression within tumors. Proc. Natl Acad. Sci. USA112, 3457–3462 (2015). CASPubMedPubMed CentralGoogle Scholar
- Krick, E. L. et al. Evaluation of Clostridium novyi-NT spores in dogs with naturally occurring tumors. Am. J. Vet. Res.73, 112–118 (2012). PubMedPubMed CentralGoogle Scholar
Acknowledgements
This work was supported by The Virginia and D.K. Ludwig Fund for Cancer Research (S.Z.), BioMed Valley Discoveries, Inc. (S.Z.), Pancreatic Cancer Action Network grant PCAN-422247 (C.G.) and US National Institutes of Health (NIH) grants CA062924 (S.Z.), CA199010 (C.G.) and GM098207 (D.B.).